A History of Fermentation
How microbiology influenced our relationship
to fermented foods
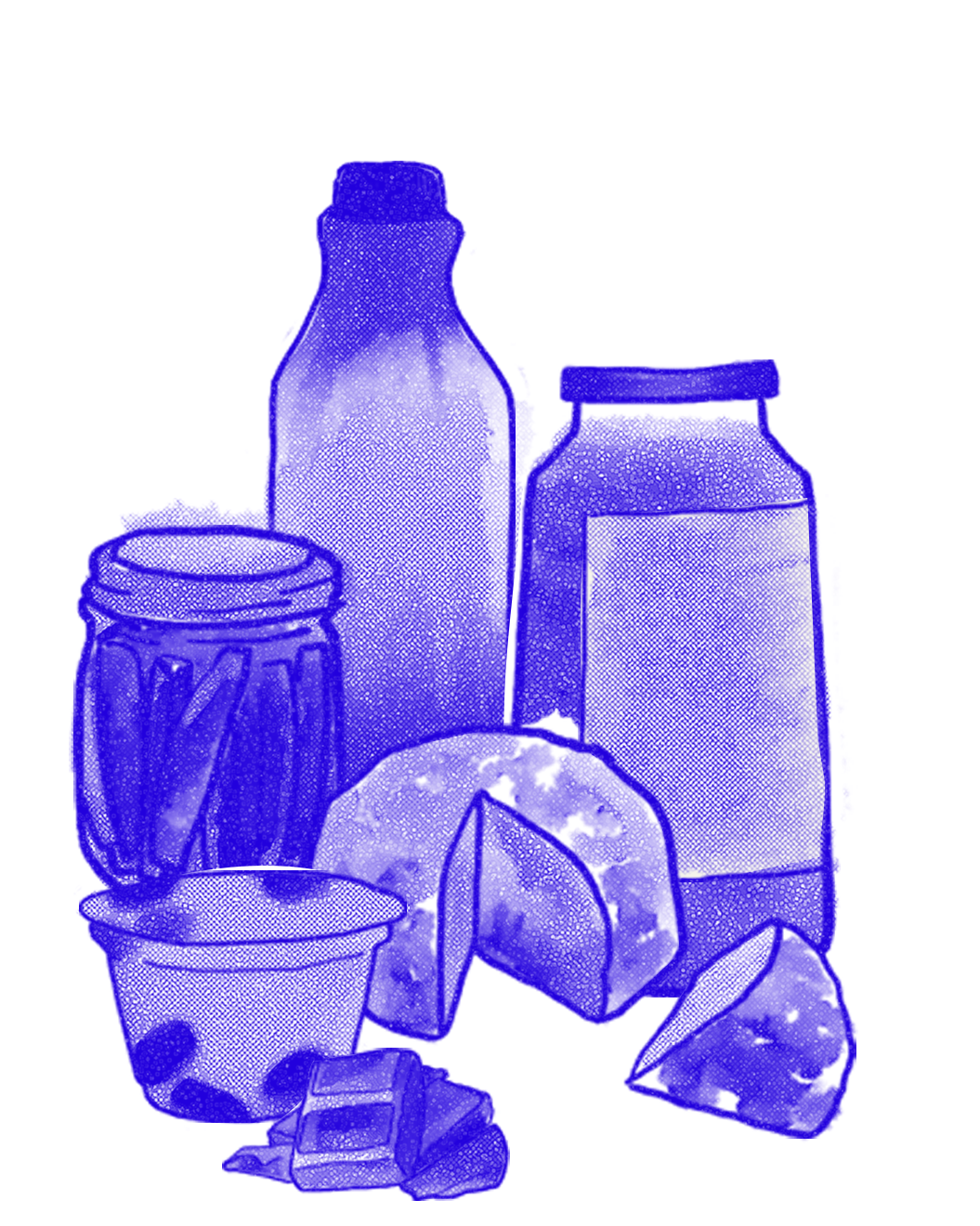
Current marketing campaigns for kombuchas or yogurts might make fermentation seem like a new practice. Far from it. Using microbes to drive “enzymatic conversions of food components” (Marco et al. 2021) has been a global practice for at least 7000 years, from early documentation of cheese, wine, and fermented grain production in Neolithic China to the commercial and home made variety of fermented foods we see today (Prajapati et al. 2003). While food fermentation has a long history and is largely regarded as safe (Adams and Mitchell, 2002), the idea of using microbes in food production is still approached with skepticism (Sahu and Panda, 2018). It is important to understand how the history of microbiology has shaped food fermentation and impacts our current relationship to fermentation production and consumption. We can then begin to define how the scientific landscape affects the production of fermented foods, identify the limitations of our current understanding of fermentation, and begin to envision the future of food fermentation.
Why ferment food to begin with? Before mass production and commercialization of fermented food (often referred to as ferments) the practice was largely for preservation (Baumgarthuber, 2021). Fermentation of vegetables like sauerkraut or kimchi allowed for continued consumption of produce throughout the winter when it otherwise would be unavailable. Cheesemaking extended the life of milks and allowed for unrefrigerated storage and transport of aged cheese. And there are additional benefits. Fermentation enhances nutrient bioavailability and can detoxify certain foods, allowing the consumer to absorb nutrients better and consume foods that might otherwise be toxic. For example, while raw cassava has naturally occurring levels of the toxin cyanide, fermenting cassava removes 90-100% of the toxin, allowing for the safe consumption of cassava and tapioca flours. Yeast-based ferments like miso or garum play a very important role in enhancing the taste of food, bringing savoriness and new flavors to the plate. And of course discussing the role of fermentation is incomplete without mentioning alcohol production, like wine and meads (Farnworth, 2008).
The beginning of the modern Western relationship to these microbially transformed foods starts with research by the French chemist Louis Pasteur, arguably one of the most important contributors to our current understanding of fermentation. In 1857 Pasteur published “Mémoire sur la fermentation appelée lactique” or “Memory on the fermentation called lactic,” where he presented how heat-treating wine prevented spoilage without compromising flavor. This eponymous process, pasteurization, involves heating food to inhibit biological agents from causing spoilage, producing off-flavors, or causing illness, informing his later work and development of modern germ theory. The discovery could not have been more timely. The rise of industrialization had resulted in more Europeans moving to large cities, translating to an increased demand for transport of food from the country to the city. Poor sanitation, lack of refrigeration, and food adulteration were common (Oblamen, 2014). The identification of microbes as the causative agent of disease, and the ability to heat-treat food to prevent illness was a remarkable advancement in food safety, a technology responsible for saving millions of lives.
It is still important to understand the limitations in scientific technology of the time. In 1857, Pasteur’s work was observational, using a microscope and tasting the wine. The now common practice in microbiology of isolating and culturing a microbe to find its identity and function was still a few years away. Even gram staining, a common method to broadly categorize bacteria based on their cell wall structure, wasn’t invented until 1884 (Coico, 2006). By studying microbes primarily in the context of preventing spoilage and food borne illness, and without the methods to isolate and characterize these strains, Pasteur unintentionally set the stage for the categorization of microbes into “good microbes,” which provide a benefit (like those necessary for food transformation through fermentation) or “bad microbes”, which include all the rest.
While the ability to characterize microbes using genetic sequencing was still a century away, it was with the invention of the petri dish and use of agar media in the 1880s that scientists began isolating and culturing these food-associated microbes. Researchers like Emil Christian Hansen began isolating yeast and creating pure cultures that could be used in beer or bread making. Rather than relying on microbes in the environment or starter cultures (like a sourdough starter) for food production, one could sterilize the ingredients first, add a lab isolated “good” microbe, and produce reliable flavor and texture. With the risk of contamination from microbes in the environment, creating a sterile environment in which to produce food reduced the risk of spoilage, allowing for a more scalable product. Marketing relied on the image of a microbe-free environment in ferment production practices (Baumgarthuber, 2021), further emphasizing the dichotomy of “good” and “bad” microbes.
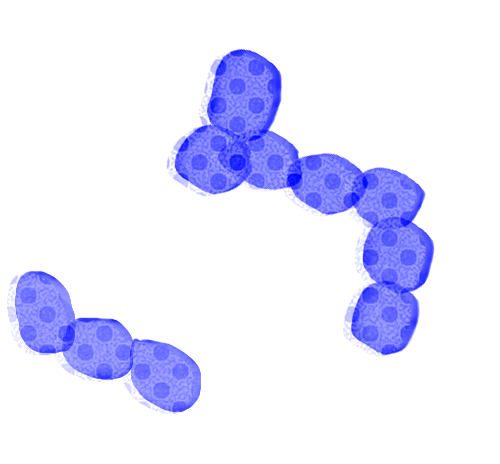
Over the next few decades, as microbiology focused on the identification and eradication of “bad” microbes, a few key advancements in science technology took place. First, the use of anaerobic chambers to isolate and grow previously unculturable microorganisms beginning in the 1940s (Wolfe, 1999). Second, the ability to derive germ-free (or microbe free) animals and colonize them with a specific set of microbes (Uzbay, 2019). And finally, sequencing technology allowed for the identification of microbes that did not grow in the culture environment, and thus had previously never been described. As it turned out, there were many more microbes in the environment, including on food and in the human body that previously believed.
While research around the microbiome continued, the real beginning of the fundamental shift away from the Pasteurian model was initiated by work published in 2006 by Jeffrey Gordon’s Lab. The experiment was fairly straightforward. Take the stool of obese mice, and transplant their stool (and along with it the microbes in their gut) into mice of normal weight. Within two weeks, the previously lean mice had significant increases in weight (Turnbaugh et al. 2006). It was the community of microbes, not a single microbe, that was contributing to the weight gain. The Pasteurian landscape was being turned on its head. The microbiome, a term used to describe an aggregate of microbes residing in a particular environment (in this example the gut microbiome, or all microbes living in the gut of the mice) showed how incomplete the previous understanding of microbes was.
How can studying the human microbiome inform our understanding of food fermentation? First, the Pasteurian way of thinking about fermented foods is reductionist. Second, thinking of fermented foods within the framework of microbial ecology, or the study of the interaction of microbes in the fermented food environment, can help us to better understand food safety, flavor profiling, and any potential health benefits. While it is true that the presence of specific pathogenic microbes has a clear impact on disease, the microbiome landscape is enormously more complex, and it is the abundance and relative presence of different microbes in the community (the diversity) that need to be considered. In fact, as of now, the only (imperfect) metric we have to measure a ‘healthy’ microbiome is diversity. The greater the diversity, the “healthier” the individual.
Although diversity is an imperfect metric, if a more diverse microbiome is positively associated with human health, what does a more diverse fermented food microbiome mean? We can first divide the current food fermentation landscape into three categories, each different in their diversity: precision fermentation, seeded fermentation, and wild fermentation. Precision fermentation is the least diverse, using microbes as a tool. Advances in synthetic biology, including commercialization of CRISPR/Cas9 technologies have allowed for the manipulation of microbes, turning them into microbial factories, where well-characterized and safe strains of yeast or bacteria can be cultured in bioreactors for the production of specific ingredients. In medicine, this had already been common practice: engineered Saccharomyces cerevisiae (brewer’s yeast) is already used for large scale production of insulin (Baeshen et al. 2014). With the rise of cellular agriculture, microbes are also engineered to produce single ingredients or flavor for food production, like heme in the burger by Impossible Foods. By focusing on fermentation as a technology, the commercial science space can scale production of single ingredients that are challenging to extract from a whole food source, or that requires the use of animal products. This means almost complete human control over what is consumed and produced by the microbes. The goal of precision fermentation is never diversity, but optimization of one metabolic product.
Seeded fermentation has relatively more diversity. It relies first on pasteurizing the starting food ingredient to create a sterile environment, followed by the addition (’seeding’) of a microbial starter typically consisting of a specific microbe or select group of microbes that will guarantee the final quality of the ferment. This is common with industrial food fermentation, thanks to its scalability and general consistency in favor and texture.
Wild fermentation relies on microbes already on the raw ingredient, in the starter, or in the environment to initiate fermentation and assemble a fermented food microbiome, and is the most diverse. However, there is more precarity to this method, less control over the final profile of the ferment. But it allows for a greater diversity of flavor, and is necessary in the case of certain fermented foods for which a reliable starter culture has yet to be developed, like in chocolate or coffee fermentation.
How does microbial diversity of fermented foods translate to health of the ferment? If we think of health as the absence of illness, food pasteurization has had remarkable success in preventing foodborne illness. Killing off any potential pathogens (and everything else) and then adding a select group of microbes means that only what you want will grow, and with fewer variables comes greater community stability. However, in the case of contamination, which might occur during processing, a contaminating microbe could enter the fermented food, and outcompete the starter strains, ruining the whole batch or even leading to human illness. This has happened before– for example, in 2009 the CDC reported a case of eight women across five states with Listeria monocytogenes poisoning caused by cheese contaminated following milk pasteurization (Jackson et al. 2011).
In wild fermentation, advances in sequencing technology have allowed a much deeper profile of the diversity of bacteria, fungi, and sometimes viruses (Lu et al. 2003; Jung et al. 2014) in ferments. While the research is just beginning, it is becoming more common to see reports of fermented foods that are consumed without causing illness contain low numbers of pathogenic strains (Leech et al. 2020). Based on our Pasteurian understanding, this should not be true. But work by researchers like Rachel Dutton, Ben Wolfe, and Paul Cotter are beginning to unravel these microbial ecological dynamics, trying to understand how the diversity of microbes might be contributing to enhanced food safety through microbial competition. In a basic microbial competition model, the diversity of wild microbes would, in simplified terms, “keep the pathogen in check” by outcompeting it for resources and secreting antimicrobial compounds (Gänzle et al. 1999; Shirako et al. 2019).
While we still do not understand how fermented foods impact health (although we do know consumption is beneficial in increasing gut microbiome diversity and reducing inflammation (Wastyk et al. 2021)) and know even less about how microbial diversity translates to “health,” we do know what tastes good and what doesn’t. With their abundant diversity and presence of microbes typically excluded from industrially produced ferments, wild fermentation promotes novel and unusual flavors produced by these microbes, a feature lost when using the same microbial starters. It is not only presence or absence of a microbe that might contribute to a final flavor or texture, but the interaction of all present that having a fermentation research space attractive to the majority of current food spaces.
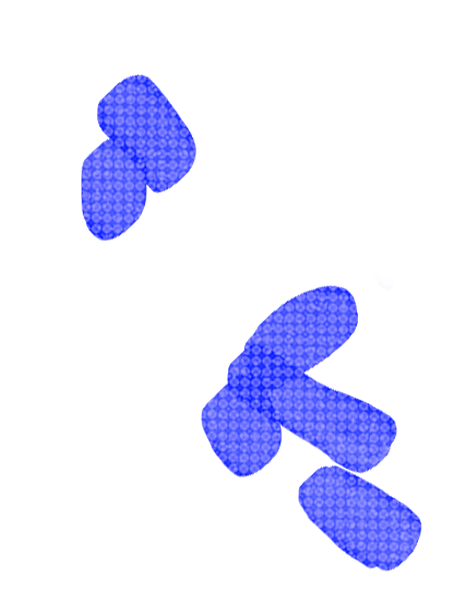
We need to abandon the Pasteruian dichotomy and embrace the ferment microbiome. While ‘wild’ fermentation seems to imply that the entire fermentation process is outside of human control, that is not true. Just like environmental factors like resource availability and climate impact how animals reproduce and compete for space, microbes depend on specific nutrient availability, water, temperature, pH, and oxygen (referred to as extrinsic factors) to maximize their fitness and dominate the fermented food. This is not a new idea in fermentation. Although it is commonly assumed that salt kills pathogens, salt actually gives halophilic (salt-loving) lactic acid bacteria (LAB) a competitive advantage over E. coli or other potential pathogens, allowing them to grow at a higher rate. As they grow and ferment, they produce anti-microbial products and lactic acid, increasing the acidity of the fermented food, and allowing LAB to now dominate the microbial community. Adding more salt inhibits growth of LAB as well, allowing for yeast to dominate the ferment instead.
None of this is novel. Controlling extrinsic factors is how food is fermented to begin with. Fermented food producers attuned to the needs of their microbes know what to look for at each step of fermentation and make changes in response. Thus, they take fermentation from a practice to a craft, shepherding microbes to create the final fermented food. This relationship had been described by scholars like Maya Hey, writing on the complexities of “multispecies communication” (Hey, 2021). The Pasteurian dichotomy has pushed microbiology research towards precision and seeded fermentation. Refocusing microbiology research towards a community ecology approach through an understanding of the microbiome has been slow to pick up, especially when it comes to fermented foods. By knowing what microbes are in the ferment and by understanding how extrinsic factors influence the final ferment, we can herd the microbial communities in our fermented foods, encouraging growth of certain microbes and discourage the growth of others without sacrificing the overall diversity of the fermented food. Using novel genomic sequencing approaches like metagenomics and by characterizing the microbial environment with proteomics and metabolomics we can begin to unravel the relationship between microbes and the final fermented food. Imagine knowing all the bacteria, yeast, and viruses present in a batch of grapes before wine fermentation begins, and knowing that although a microbe responsible for a specific fault is present, all you need to do is lower the temperature after the first week. Or imagine, in a batch of sauerkraut, knowing that addition of extra salt will increase the presence of a specific LAB strain that adds a depth of flavor. No need to pasteurize or sterilize the microbial landscape. We can maintain microbial diversity without risking safety or faults or spoilage, all the while promoting abundance of texture and flavor.
It’s ironic that Pasteur unintentionally directed the field towards focusing on microbes as foes (or neural players at best), when his name comes from the Latin pastōrem, or shepherd. His initial experiments in pasteurization were not focused on preventing illness but on preventing the spoilage of fermented foods, focusing on wine (especially important for him coming from the town of Arbois in the French Jura wine region), vinegar, and cheese. And while he was limited by technology for his understanding of microbial life, we are not. By embracing the complexities of the microbial landscape, we will be able to see beyond the role of individual microbes and change our relationship to food fermentation. Beyond antimicrobial agents, texture, and flavor, who knows what fermented landscapes might be a source for? The field is wide open.
References
- Adams, Martin, and Robert Mitchell. "Fermentation and pathogen control: a risk assessment approach." International Journal of Food Microbiology 79.1-2 (2002): 75-83.
- Baeshen, Nabih A., et al. "Cell factories for insulin production." Microbial cell factories 13.1 (2014): 1-9.
- Baumgarthuber, Christine. Fermented Foods: The History and Science of a Microbiological Wonder. Reaktion Books, 2021.
- Coico, Richard. "Gram staining." Current protocols in microbiology 1 (2006): A-3C.
- Farnworth, Edward R. Ted. Handbook of fermented functional foods. CRC press, 2008.
- Gänzle, Michael G., Sigrid Weber, and Walter P. Hammes. "Effect of ecological factors on the inhibitory spectrum and activity of bacteriocins." International journal of food microbiology 46.3 (1999): 207-217.
- Jackson, K. A., et al. "Multistate outbreak of Listeria monocytogenes associated with Mexican-style cheese made from pasteurized milk among pregnant, Hispanic women." Journal of food protection 74.6 (2011): 949-953.
- Jung, Ji Young, Se Hee Lee, and Che Ok Jeon. "Kimchi microflora: history, current status, and perspectives for industrial kimchi production." Applied microbiology and biotechnology 98.6 (2014): 2385-2393.
- Leech, John, et al. "Fermented-food metagenomics reveals substrate-associated differences in taxonomy and health-associated and antibiotic resistance determinants." MSystems 5.6 (2020): e00522-20.
- Lu, Zhong Jing, et al. "Bacteriophage ecology in commercial sauerkraut fermentations." Applied and Environmental Microbiology 69.6 (2003): 3192-3202.
- Marco, Maria L., et al. "The International Scientific Association for Probiotics and Prebiotics (ISAPP) consensus statement on fermented foods." Nature Reviews Gastroenterology & Hepatology 18.3 (2021): 196-208.
- Obladen, Michael. "From swill milk to certified milk: progress in cow's milk quality in the 19th century." Annals of Nutrition and Metabolism 64.1 (2014): 80-87.
- Paxson, Heather. "Post-Pasteurian cultures: The microbiopolitics of raw‐milk cheese in the United States." Cultural anthropology 23.1 (2008): 15-47.
- Prajapati, Jashbhai B., and Baboo M. Nair. "The history of fermented foods." Handbook of fermented functional foods (2003): 1-25.
- Uzbay, Tayfun. "Germ-free animal experiments in the gut microbiota studies." Current Opinion in Pharmacology 49 (2019): 6-10.
- Sahu, Lopamudra, and Sandeep Kumar Panda. "Innovative technologies and implications in fermented food and beverage industries: An overview." Innovations in technologies for fermented food and beverage industries (2018): 1-23.
- Shirako, Saki, et al. "Pyroglutamyl leucine, a peptide in fermented foods, attenuates dysbiosis by increasing host antimicrobial peptide." NPJ science of food 3.1 (2019): 1-9.
- Turnbaugh, Peter J., et al. "An obesity-associated gut microbiome with increased capacity for energy harvest." nature 444.7122 (2006): 1027-1031.
- Uzbay, Tayfun. "Germ-free animal experiments in the gut microbiota studies." Current Opinion in Pharmacology 49 (2019): 6-10.
- Wastyk, Hannah C., et al. "Gut-microbiota-targeted diets modulate human immune status." Cell 184.16 (2021): 4137-4153.
- Wolfe, Ralph S. "Anaerobic life—a centennial view." Journal of bacteriology 181.11 (1999): 3317-3320.